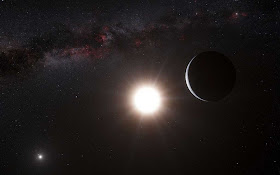 |
This artist’s impression shows the planet orbiting the star Alpha
Centauri B, a member of the triple star system that is the closest to
Earth. Alpha Centauri B is the most brilliant object in the sky, and the
other dazzling object is Alpha Centauri A. Our own Sun is visible to
the upper right. The tiny signal of the planet was found with the HARPS
spectrograph on the 3.6-meter telescope at ESO’s La Silla Observatory in
Chile. // Credit: ESO/L. Calçada/N. Risinger |
The observations extended over more than four years using the HARPS
instrument and have revealed a tiny signal from a planet orbiting Alpha
Centauri B every 3.2 days.
European astronomers have discovered a planet with about the mass of the
Earth orbiting a star in the Alpha Centauri system — the nearest to
Earth. It is also the lightest exoplanet ever discovered around a star
like the Sun. The planet was detected using the HARPS instrument on the
3.6-meter telescope at the European Southern Observatory’s (ESO) La
Silla Observatory in Chile.
Alpha Centauri is one of the
brightest stars in the southern sky and is the nearest stellar system to
our solar system, only 4.3 light-years away. It is actually a triple
star — a system consisting of two stars similar to the Sun orbiting
close to each other, designated Alpha Centauri A and B, and a more
distant and faint red component known as Proxima Centauri. Since the
19th century, astronomers have speculated about planets orbiting these
bodies, the closest possible abodes for life beyond the solar system,
but searches of increasing precision had revealed nothing. Until now.
"Our
observations extended over more than four years using the HARPS
instrument and have revealed a tiny, but real, signal from a planet
orbiting Alpha Centauri B every 3.2 days," said Xavier Dumusque from the
Geneva Observatory in Switzerland and the University of Porto in
Portugal. "It's an extraordinary discovery, and it has pushed our
technique to the limit!"
The European team detected the planet by
picking up the tiny wobbles in the motion of the star Alpha Centauri B
created by the gravitational pull of the orbiting planet. The effect is
minute. It causes the star to move back and forth by no more than 20
inches (51 centimeters) per second, about the speed of a baby crawling.
This is the highest precision ever achieved using this method.
Alpha
Centauri B is very similar to the Sun but slightly smaller and less
bright. The newly discovered planet, with a mass of a little more than
that of Earth, is orbiting about 3.7 million miles (6 million
kilometers) away from the star, much closer than Mercury is to the Sun
in the solar system. The orbit of the other bright component of the
double star, Alpha Centauri A, keeps it hundreds of times farther away,
but it would still be a brilliant object in the planet's skies.
This
same team found the first exoplanet around a Sun-like star in 1995, and
since then there have been more than 800 confirmed discoveries, but
most are much bigger than Earth, and many are as big as Jupiter. The
challenge astronomers now face is to detect and characterize a planet of
mass comparable to Earth that is orbiting in the habitable zone around
another star. The first step has now been taken.
"This is the
first planet with a mass similar to Earth ever found around a star like
the Sun. Its orbit is very close to its star, and it must be much too
hot for life as we know it," said Stephane Udry from the Geneva
Observatory, "but it may well be just one planet in a system of several.
Our other HARPS results and new findings from Kepler both show clearly
that the majority of low-mass planets are found in such systems."
"This
result represents a major step towards the detection of a twin Earth in
the immediate vicinity of the Sun. We live in exciting times!" said
Dumusque.